Extreme weather events such as rainfall and drought are governed by complex weather systems and interactions between the ocean, atmosphere and land. Understanding the drivers behind extreme weather events is important to help prepare for and, as far as possible, mitigate or respond to the impacts of those events.
Predicting future climate scenarios carries a degree of uncertainty because the ways in which weather systems may change in response to climate change will depend upon complex interactions on different timeframes. A key factor that climate modellers must consider is the quantity of anthropogenic greenhouse gases that will be released into the atmosphere in future decades. That quantity is dependent upon future human behaviour – and that in itself is unpredictable.
Facing the Weather Gods highlighted that understanding of the drivers of African climate identified below is poor, but what has happened in the intervening years? Overall, the weather systems are becoming better understood but more research is still required; additional observational data will help to reduce uncertainties in modelling predictions. Climate forecasts are improving because of new observational systems, particularly those that are satellite based, and advances in computing can incorporate detailed physical, chemical and biological processes (IPCC, 2013).
Three main weather drivers were addressed in Facing the Weather Gods: the Inter-Tropical Convergence Zone (ITCZ); the West African Monsoon (WAM); and the El-Niño Southern Oscillation (ENSO) (already summarised in Box 4). These three major systems are revisited here, as well as other major global systems that influence Africa’s weather. Possible future impacts of climate change on the main weather systems are discussed in the sections below.
3.1 The main drivers discussed in Facing the Weather Gods
As mentioned in Facing the Weather Gods, the Atlantic, Pacific and Indian oceans are instrumental in the formation of weather patterns and driving climate variability, although the relationships between the oceans and weather patterns need more research to be fully understood. In the tropical regions of the continent, the monsoon system dominates the climate. The dominant driver of East African rainfall is the sea surface temperature of the Indian Ocean, although the semi-annual rainfall over East Africa is driven by the ITCZ, and the ENSO also influences periods of drought and precipitation. Southern Africa is highly influenced by the oceans and ENSO (Christensen et al., 2013, section 14.8.7).
3.1.1 Inter-Tropical Convergence Zone (ITCZ)
As summarized in the Facing the Weather Gods report:
“The Intertropical Convergence Zone (ITCZ) appears on satellite images as a band of clouds, often thunderstorms which encircle the globe near the equator. These clouds are formed by air convection driven by solar heating together with the converging trade winds. As this air rises it cools and leads to intense rainfall on an almost daily basis. The ITCZ moves seasonally over land as a result of the tilt of the Earth’s axis and the changing solar zenith. It moves north in the northern summer and south in the northern winter (for an animation of the seasonal migration of the ITCZ, see ITCZ, 2020). This drives the seasonality of rainfall in Africa and the ITCZ is largely responsible for rainfall occurring between latitudes of approximately 23.5° N and S. The distance that the ITCZ moves seasonally is governed in part by differences in land and sea temperatures, and it moves a greater distance southwards in the central to eastern areas of the continent. As the ITCZ crosses areas twice, it gives rise to two wet periods. The degree of latitudinal movement also varies from year to year explaining some of the inter-annual variation in rainfall.”
East Africa
East Africa, as defined in Nicholson (2017), includes Kenya, Uganda, Tanzania, Ethiopia, Eritrea, Djibouti, Somalia, South Sudan, Rwanda and Burundi (though historically, many researchers have regarded East Africa as Uganda, Kenya and Tanzania alone). Although climatology is highly variable across East Africa, the wider region described here includes the areas of East Africa which have two rainy seasons each year.
At present, the mean annual rainfall over East Africa is between 800 and 1,200 mm, with more arid and humid extremes in northern and highland regions respectively. Precipitation patterns over the region are complex and vary depending on the topography, season and also from year-to-year. Of the two rainy seasons, the long rains over East Africa fall in the period March–April–May, and produce more rainfall than the short rains (which fall during October–November), which are also less reliable. The main climate trends are that the long rains appear to have declined over much of East Africa and the short rains have increased, periods of drought have become longer and more intense and sometimes continue through rainy seasons, and severe floods have affected the region (Nicholson, 2017).
Rainfall patterns and variability over East Africa can broadly be explained by the seasonal migration of the ITCZ but research suggests that zonal winds over the central equatorial Indian Ocean are a complicating factor. On an inter-annual time scale, the El-Niño Southern Oscillation (ENSO) and the Indian Ocean Dipole (IOD) are also important (Nicholson, 2017).
The ITCZ is a band of deep convective clouds – that is, clouds that can be up to 10km thick – that form across the tropics. The ITCZ shifts seasonally towards the hemisphere that is warmer in relation to the other but the precise mechanisms that control its position, and rainfall intensity, are unclear. Small changes in the atmospheric energy balance between the tropics and extratropics can lead to substantial ITCZ migrations. Consequently, climate modelling projections of future ITCZ movements are very uncertain. Future research to understand the processes that affect the position of the ITCZ will help climate modelling to project the movement of the ITCZ in the future (Schneider et al., 2014).
On the basis that the ITCZ influences rainfall patterns, understanding the mechanisms that influence the position of the ITCZ could be important in projecting East Africa’s future climate scenario.
A number of climate systems (in addition to the ITCZ) influence rainfall over Africa, and the relationship between East Africa’s rainfall patterns and global weather systems is complex. Although the influence of ocean surface water cooling/ warming on rainfall patterns is not yet fully understood, there does seem to be a relationship between sea surface temperatures in the Indian Ocean and precipitation. In addition, the variation in East Africa’s short rains seem to be related to the Indian Ocean Dipole. The Indian Ocean Dipole (more so than the ENSO) seems to be more influential over variability of East Africa’s short rains than over the region’s long rains (Nicholson, 2017).
The atmosphere above East Africa is generally stable but the rainy seasons (March–April–May and October–November) coincide with increased sea surface temperature in the western Indian Ocean, which transports less-stable air to east Africa, bringing rains as the ITCZ passes over (Tierney et al., 2013; Ummenhofer et al., 2018). As the ITCZ continues its seasonal migration, the rains in Central Africa and the western highlands of East Africa arrive in June–September.
As the Indian Ocean has warmed, there has been a noticeable decrease in rainfall over West and Central Africa. The mechanism for this could be the presence of a strong Rossby wave that leads to a moisture deficit in the region, but also because of the ITCZ. The suggestion is that the decline in rainfall over West and Central Africa is because of a weakened Atlantic ITCZ, and the increase in rainfall over East Africa is a result of a strengthened Indian Ocean ITCZ. As warming intensifies in the Indian Ocean, the expectation is that the trends in rainfall response over Central and East Africa will also intensify – that is, the Central African region will continue to become drier, and the trend over East Africa will be for increased rainfall during the short rains (Dhame et al., 2020).
In summary, observed precipitation trends show drying over Central Africa and the Sahel (Dhame et al., 2020), and wetter short rains (October–November) and drier long rains (March–April–May) over the Horn of Africa (Liebmann et al., 2014).
Declining rains are of great consequence for agriculture, and therefore research to predict more accurately how rainfall patterns might change could help in the development of contingency plans for potential periods of drought. However, because rainfall patterns vary naturally on decadal timescales, it is important to note that a drought in one particular year could be caused by factors other than anthropogenic climate change. One theory for the precipitation changes, based on modelling predictions from a 40-member ensemble of ECHAM5 sea surface temperature-forced atmospheric simulations, suggests that the drier long rains (March–April–May) are because of increased sea surface temperatures in the ocean between Indonesia and the central Pacific, and the wetter autumn rains (October–November) are mostly attributed to increased sea surface temperature in the western Indian Ocean (even though the long rains are strongly correlated with ENSO and IOD) (Liebmann et al., 2014).
The ITCZ seems to be just one of a number of weather systems that influence rainfall patterns over East Africa. The reasoning is partly explained by the observation that the ITCZ is much further north during the equatorial rainy season (Nicholson, 2018). So although the ITCZ is an important driver of the seasonal rainfall over East Africa, research suggests that the ITCZ alone does not account for all the rainfall conditions in that region. The sea surface temperature in the western Indian Ocean influences the two rainy seasons, as does the ENSO (Yang et al., 2015; Nicholson, 2017; Nicholson, 2018). More research is needed to determine the extent of the relationship between East Africa’s seasonal rains and the ITCZ.
3.1.1.1. IPCC AR5 and the ITCZ
Modelling (based on a CMIP5 multi-model ensemble of 18 models) under RCP8.5 suggests an increase by around 1.6mm per day rainfall associated with the ITCZ by the 2080s (Christensen et al., 2013, section 14.3.1.1).
3.1.2 West African Monsoon
The West African Monsoon is typically associated with wet summers (July–September) and drought in the winters. Unlike some other weather systems, the West African Monsoon is associated with variable rainfall. It is useful to consider the mechanisms of the West African Monsoon. The land warms faster than the ocean during the summer months, causing the ITCZ to migrate northwards, bringing cool air and rain. The ITCZ pushes back the warm northeasterly trade winds, also called the Harmattan. The ITCZ migrates southwards, towards equatorial Africa, by October, and the trade winds return (Cornforth, 2012).
Facing the Weather Gods included the following description of the West African Monsoon:
“The West African Monsoon (WAM) is another important climate driver in Africa. The heating of the land causes air above the Sahara to rise, drawing warm moist air in from the sea 1000km to the south. This south westerly airflow then generates rainfall over parts of West Africa from April to June. In mid-July, the rainfall maximum moves suddenly northwards following the movement of the ITCZ. This movement is related to easterly atmospheric waves, which in turn are associated with the ITCZ. The precise relationships are not well characterised.”
The West African Monsoon brings varying quantities of rainfall on timescales ranging from annual to multidecadal. Global sea surface temperature rise and the increase in atmospheric CO2 are key drivers for change and variability in the West African Monsoon, according to analysis involving 12 atmospheric-ocean coupled models in the CMIP5 archive. Quantifying the impacts of CO2 forcing (global heating) on the West African Monsoon still needs further research (Gaetani et al., 2017).
The West African Monsoon is an important water source for the Sahelian countries, bringing 80% of the rain from late June to late September. Fluctuations in the rainfall can arise because of changes in the wind and relative humidity. As the climate warms, the associated changes to weather systems may introduce greater uncertainties in projecting future weather using climate modelling. Multi-model analysis of CMIP5 and CMIP6 (an analysis of many climate model simulations) found that models are not consistent in their projections of how rainfall patterns over the Sahel may change in future decades and this is likely to be because, as the climate warms, the atmospheric circulation will change in ways that are currently difficult to model consistently. To date, different models have produced appreciably different end scenarios (Monerie et al., 2020).
The possible impact of climate-driven changes to the West African (and Indian) Monsoon on rainfall in East Africa, upon both summer rainfall and to the early and late months of the long and short rains, has so far been little studied (Nicholson, 2017).
3.1.2.1 IPCC AR5 and the West African Monsoon
The IPCC AR5, published since Facing the Weather Gods, provided valuable additional information, but much more has been published in the scientific literature since then. The next major IPCC review, AR6, is scheduled to be published in 2021 and 2022. AR5 draws on Coupled Model Intercomparison Projects (CMIP3 and CMIP5) but a more up-to-date modelling project, CMIP6, exists. Briefly: AR5 (Flato et al., 2013, section 9.5.2.4) found that while CMIP5 models better simulate monsoon climatology in comparison to CMIP3, the regional scale quality needed improving. As noted above, in relation to the West African Monsoon, different models project different outcomes, which highlights, for example, the difficulty in simulating the response of the African rain belt to anomalies in sea surface temperatures in the Atlantic (Flato et al., 2013, section 9.5.3.5.2). There is only low confidence in the accuracy of models predicting rainfall over West Africa (Christensen et al., 2013, sections 14.2.4, 14.8.7). Regarding temperature, ten regional climate models run for Africa overestimated the daily minimum temperature (Flato et al., 2013, section 9.6.3), again highlighting the overall uncertain nature of projecting changes to the climate (Flato et al., 2013).
In summary, future projections for the West African Monsoon outlined in the IPCC AR5 section on monsoons suggest that as global temperatures rise, monsoons will become more intense and affect a wider area as higher temperatures increase the moisture content of the air. That said, future regional trends in the intensity and timing of monsoons are uncertain in many parts of the world. Also, no long-term trends have been observed in the West African Monsoon and future projections of monsoon rainfall are unclear not least because of uncertainties in the model projections (Christensen et al., 2013, section 14.2.4).
3.1.3 El-Niño Southern Oscillation (ENSO)
Facing the Weather Gods acknowledged that understanding of the mechanisms and likely future behaviour of the El-Niño Southern Oscillation (ENSO) was poor, as was knowledge of its interactions with other climate drivers. How has research over the past seven years helped to advance understanding of the influence that ENSO has on African weather?
The ENSO influences extreme weather events globally, causing floods in some regions and droughts in others (Cai et al., 2018). Most of the severe droughts in Southern Africa have been associated with ENSO, and since the 1970s the influence of ENSO on the Southern African climate has become stronger (Rouault & Richard, 2005). The ENSO is a naturally occurring oscillating interaction between the tropical Pacific Ocean and the atmosphere and comprises so-called El Niño and La Niña phases. In summary, El Niño occurs every three to seven years and brings warm, dry air to Southern Africa and cool air and rain to eastern equatorial Africa. The opposite happens in La Niña years – cool air and rain are brought to Southern Africa and warm, dry air to equatorial East Africa.
The ENSO is a driver of year-on-year rainfall differences over East Africa with El Niño/La Niña years resulting in wet/dry conditions during the short rains (October-November). The impact of El Niño or La Niña on rainfall seems to depend on the temperature of the Indian and Atlantic Oceans at the time (Nicholson, 2017). The climatic impact of ENSO is slightly different in southeast Africa – the general scientific consensus is that El Niño years are drier and during La Niña years the conditions are wetter. However, the relationship between ENSO and rainfall over Southern Africa is complex and anomalies exist, possibly because of the variation in sea surface temperature in the Atlantic, Pacific and Indian Oceans during ENSO events (Hoell et al., 2015; Gore et al., 2020).
Work to understand the role of the ENSO has identified eight ENSO sea surface temperature patterns that influence rainfall over Southern Africa. During El Niño events, warmer than average sea surface temperature in the Atlantic and Indian oceans is associated with decreased rainfall over Southern Africa; El Niño with cooler than average sea surface temperature over the Indian Ocean is associated with increased rainfall over Southern Africa. La Niña is generally associated with a negative Indian Ocean Dipole (Lim & Hendon, 2017) – with increased precipitation over Southern Africa and decreased precipitation over East Africa. Understanding the behaviour of ENSO and associated systems will help to predict future periods of extreme weather over African regions (Hoell et al., 2015).
The ENSO has also been associated with elevated temperature over Southern Africa. Temperature affects evapotranspiration which can, in turn, impact on drought. One study that used ten regional climate models concluded that the connection between ENSO and drought conditions is because ENSO influences both rainfall and temperature (Meque & Abiodun, 2015).
Fast-forward to future decades, and the concern is that East African countries may experience an overall decline in rainfall as a result of climate change. In comparison to the twentieth century (reference period 1976–2005), projections for the end of the century (2070–2100) suggest a decrease in the mean rainfall over the region during the June–September and March–May seasons, and an increase in rainfall over equatorial and southern part of the region during October–December. The largest changes are projected in the equatorial region (Endris et al., 2019). Unresolved issues with climate models have led to conflicting findings in some areas. For example, while modelling consistently projects increased rainfall over East Africa from the long rains, observational data show a decline from the late 1980s through to the late 2000s. This is known as the East African Climate Paradox and appears to be due to a shortening of the rainy season (with later onset and earlier cessation) rather than by a decrease in the peak daily rainfall. In turn, this may be due to interactions between a variety of factors. Such issues need to be resolved before predictions are sound enough to inform decisions about adaptive strategies (Wainwright et al., 2019).
3.1.3.1 IPCC AR5 and the ENSO
The ENSO varies on an inter-decadal timeline. Models do not seem to agree on whether the observed changes in ENSO are because of anthropogenic changes or natural variability. The IPCC AR5 reports high confidence that the ENSO will remain the dominant mode of interannual variability with global influences through the twenty-first century, and that rainfall associated with the ENSO will become more intense (Christensen et al., 2013, section 14.4.4).
Since the IPCC’s AR5 was published, a re-analysis of climate models that assess the impact of global heating on ENSO conclude that there will be an increase in sea surface temperature variance in the equatorial Pacific. The increased sea-surface temperature variance in the eastern Pacific during El Niño is attributed to climate change. The increased sea temperature will further drive strong equatorial Pacific El Niño events and, in turn, is expected to lead to an increase in extreme weather events in other ocean areas that can cause floods and droughts including in Latin America and the Caribbean (Cai et al., 2018).
3.2 Other meteorological systems relevant to extreme weather in Africa
3.2.1 The Mascarene High
The Mascarene High is a semi-permanent anticyclone (a weather system associated with calm weather) located above the Southern Indian Ocean, which undergoes strong inter-annual variations and influences weather patterns over Southern Africa and Australia (Vidya et al., 2020). Changes in the atmospheric circulation system due to climate change, such as the poleward migration of the Mascarene High, is highly likely to change the weather systems over Southern Africa, which may lead to shifts in the subtropical dry zones and significant changes in mid-latitude rainfall. Severe weather events such as floods and extreme cold can arise from atmospheric ‘blocking’ caused by the Mascarene High. A simplified explanation of this complex system is that the ‘blocking’ action of the Mascarene High is an anomaly that slows down the west–east ocean–atmosphere circulations over Southern Africa, which can mean, in turn, that unstable weather remains over a region for an extended period. An example was the weather system that affected eastern South Africa (Durban) in April 2019 when flooding killed at least 85 people and displaced thousands of others.
The Mascarene High also helps determine the path taken by tropical cyclones over the Mozambique Channel and Southern Africa, storms which can cause widespread destruction when they make landfall. According to Xulu et al. (2020), understanding weather systems such as the Mascarene High will help local societies to predict and plan adaptations for future weather events and reduce the loss of human life from tropical cyclone landfalls. The part played by the Mascarene High in future climate scenarios does not seem to have been extensively researched and, therefore, warrants further investigation.
3.2.2 Turkana low-level jet
The Turkana low-level jet blows through the Turkana Channel, a 700‐km long stretch of low lying land between the Ethiopian and East African highlands in northern Kenya. The jet is a wind that regularly achieves 30 m s−1, but occasionally reaches 60 m s−1, and which is generally stronger at night time. The Turkana low-level jet was discovered in the 1980s but has still not been well studied, though it is thought to be involved in aridity in northeast Kenya, southern Somalia and southeastern Ethiopia, and rainfall variability in the East Africa region. The Turkana low-level jet may inhibit summer rains in northwest Kenya. Low-level jets in other regions of the world (such as the Great Plains of the central United States and the La Plata basin in South America) are associated with extreme rainfall. Changes to the Turkana low-level jet that occur as a result of global heating could affect rainfall patterns and, in turn, local agriculture and the economy. However, more studies are needed to assess the extent of influence of the Turkana jet on aridity and rainfall (Hartman, 2018; Nicholson, 2016).
3.2.3 Madden–Julian oscillation
This phenomenon is an area of tropical rain that is thought to partly influence the intensity of El Niño and La Niña events. The weather system is associated with increased rainfall in equatorial regions that operates on a 40–50 day cycle. Research indicates that the rainfall associated with the Madden–Julian oscillation will likely increase in intensity with anthropogenic climate change, but more research is needed to project the likely extent of the changes to rainfall, and to understand the influence on the oscillation from sea surface temperature fluctuations (Maloney et al., 2019).
3.2.4 Walker circulation
The Walker circulation is an atmospheric circulation pattern over the equatorial Indian and Pacific oceans. The Walker circulation is associated with ENSO and the two weather systems seem to be influential in rainfall patterns over East Africa (King et al., 2019).
3.3 Indian Ocean Dipole
The Indian Ocean Dipole refers to the sea-surface temperature difference on the two sides of the Indian Ocean. A positive Indian Ocean Dipole means that sea temperatures are warmer in the western Indian Ocean region and cooler in the east, bringing heavy rainfall to East Africa. A negative dipole is the opposite and brings drier conditions to East Africa. The most extreme recent strong negative Indian Ocean Dipole in 2016 was a key driver of the weak La Niña and the conditions contributed to the prolonged drought over East Africa that severely affected the livelihoods of more than 15 million people at the end of 2016 (Lu et al., 2018). The Indian Ocean Dipole also impacts weather systems further afield: in Australia, a positive dipole means less rain in the south and far north of the country, and a negative dipole means the opposite with more rainfall in the far north and over the southern region.
Hence, warm temperatures in the western Indian Ocean and cooler temperatures in the east Indian Ocean lead to high rainfall and flooding events in East Africa and droughts and fires in west Australia and southeast Asia because the rain ‘follows’ the warmer sea water. The extreme weather events associated with the Indian Ocean Dipole on two different continents have been widely reported in the mainstream global media (for example: Uchoa, 2019). The phenomenon is exacerbated if the dipole is strongly positive or higher than average, as happened in 1961, 1994, 1997 and 2019. Floods this year that affected an estimated six million people across East Africa, in Somalia, Ethiopia, Burundi, Djibouti, Kenya, Rwanda, Somalia, Tanzania and Uganda (BBC, 2020), have been attributed to extreme rainfall linked to the positive Indian Ocean Dipole.
The Indian Ocean comprises around 12% of the global ocean area, and yet has absorbed 28% of global ocean heat gain, which means that the Indian Ocean seems to absorb heat disproportionately to elsewhere. Measurements taken from 1960–2015 show that the upper 2,000m (but especially the uppermost 300m) of the Indian Ocean have undergone consistent warming and have warmed by 1.04 °C over the measurement period. More than 90% of the surface warming is attributed to anthropogenic greenhouse gas emissions (Collins et al., 2019, section 6.5.1.2). Projected changes to the Indian Ocean Dipole differ between the different models, but modelling under RCP8.5 indicates (with low confidence) an increase by a factor of three in the frequency of extreme positive IOD events. That translates to one event every 6.3 years over the twenty-first century, which would have serious implications for East African rainfall patterns (Cai et al., 2014).
Box 5: Humans as climate drivers
The connection between atmospheric carbon dioxide levels and the Earth’s climate has been known about for more than 120 years. In 1896, the Swedish physicist/chemist Svante Arrhenius (who was awarded Nobel prize in chemistry in 1903) was the first person to quantify the warming effect of carbon dioxide on the Earth’s atmosphere. Arrhenius undertook what he refers to in his paper as ‘tedious calculations’ and concluded that an increase in atmospheric CO2 would have a warming effect on the Earth’s atmosphere – for example, he noted that the temperature would rise in the Arctic regions by 8 ℃ or 9 ℃ if the CO2 rose 2.5 or 3 times that of the (then) present level (Arrhenius, 1896). Modern observations show that the Arctic has warmed substantially since 1950 and warming is predicted to continue in the future, far exceeding the average global warming rate (Xu et al., 2013). Predictions are that by the end of the century, in comparison to the present day the Arctic will be 7 ℃–13 ℃ warmer in the autumn and 3 ℃–5 ℃ warmer in the spring (Overland et al., 2013). Although he received criticism at the time, Arrhenius’s thinking was ahead of his time.
In the 1970s, scientists predicted that if atmospheric carbon dioxide levels doubled in comparison to pre-industrial levels, largely as a result of the burning of fossil fuels, then the global temperature increase would be in the range 1.5 ℃–4.5 ℃ – a range known as ‘climate sensitivity’. In other words, climate sensitivity is the potential increase in temperature if anthropogenic carbon dioxide emissions continue until they are double that of the pre-industrial period. An analysis of many studies that were published during the past 20 years have predicted the same range in climate sensitivity that scientists predicted in the 1970s (IPCC, 2014a).
Analysis of 17 climate model projections published between 1970 and 2007 found that climate models over the past five decades have been “generally quite accurate” in predicting changes to global mean surface temperature (Hausfather et al., 2020).
The IPCC claimed in 1990 that if atmospheric CO2 levels doubled that of pre-industrial times, the likely annual global temperature increase is 1.5 ℃–4.5 ℃, which is the same predicted temperature range in the most recent report, AR5.
The IPCC was set up in 1988 and published its first report in 1990 (IPCC, 1990). That report said that to stabilize long-lived gases (CO2, N2O, CFCs) at the then-present-day levels would mean reducing anthropogenic emissions of those gases by more than 60%. In 1990, average carbon dioxide concentration at Earth’s surface was 353 parts per million (ppm). It also says that “episodes of high temperatures will most likely become more frequent in the future, and cold episodes less frequent”. The report states an accepted climate sensitivity range from models of 1.5 ℃–4.5 ℃ (IPCC, 1990; Policymakers Summary, p. xxv).
When is it likely that atmospheric carbon dioxide levels will reach double that of the pre-industrial era? In the mid-1700s, the pre-industrial period, the level of atmospheric CO2 was around 280 ppm. In 2019, the atmospheric CO2 was 409.8 ± 0.1 ppm, an increase of almost 50%. The present atmospheric CO2 concentration is at its highest for the past 800,000 years (Blunden & Arndt, 2020) (Fig. 2). Using data from ice cores, it is possible to analyse ancient atmospheric CO2 levels. For example, 30,000 years ago the estimated CO2 concentration in the atmosphere was 190ppm (Our World in data; https://ourworldindata.org/atmospheric-concentrations; NOAA https://www.climate.gov/news-features/understanding-climate/climate-change-atmospheric-carbon-dioxide)
Fig. 2a shows a reconstruction of Antarctic temperature over the past 800,000, and Fig. 2b shows reconstructed CO2 concentrations over the same time period. The striking similarity between the two curves shows how closely atmospheric CO2 concentrations have correlated with temperature, and hence with climate (using Antarctica as a proxy for global climate).
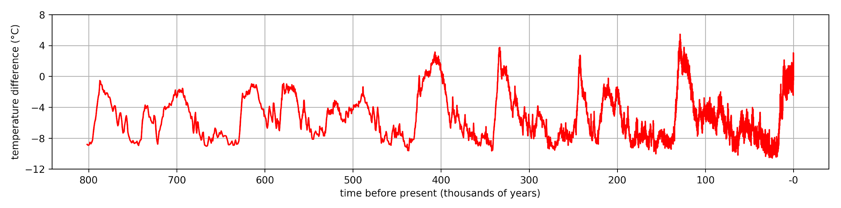
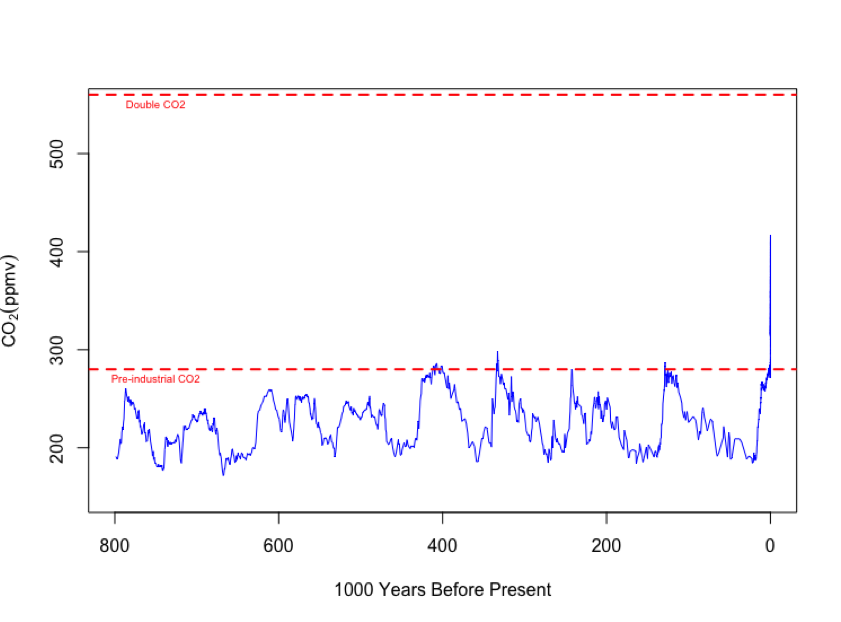